The fungi in our guts can make cases of Covid worse
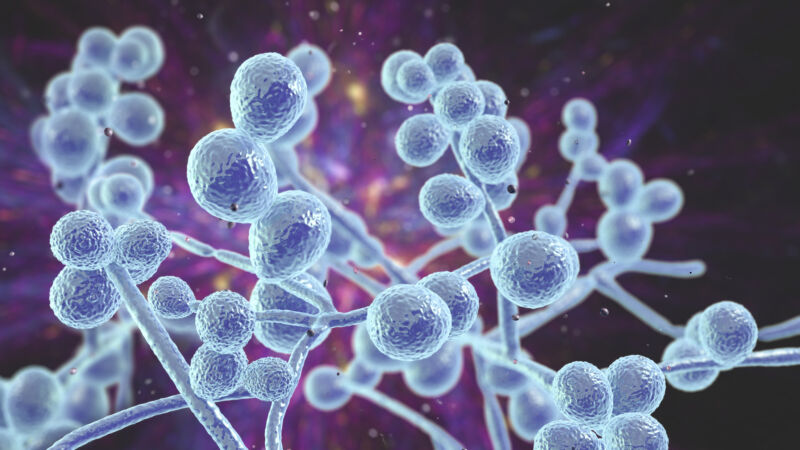
Enlarge / Computer illustration of Candida fungi.
Kateryna Kon | Science Photo Library | Getty
Fungi are an indispensable part of your microbiome, keeping the body’s host of microorganisms healthy as part of a system of checks and balances. But when you’re hit by an infection, fungi can be thrown out of equilibrium with other organisms inside you, leading to a more severe infection and other symptoms of illness.
For this reason, the pandemic immediately set off alarms for Iliyan Iliev, an immunologist at Weill Cornell Medical School. “We were thinking, the first thing that’s going to happen is people will start getting fungal co-infections,” he says. With the microbiome unbalanced, fungi might start running riot inside Covidpatients, Iliev reasoned. His fears were soon realized.
In research published in Nature Immunology, he and his team discovered that in patients with severe Covid, certain strains of gut fungi—knocked off-kilter by the virus—set off a prolonged immune response that could last long after the initial infection. This response potentially led to some of the respiratory symptoms experienced by these patients. These results, Iliev says, point to the critical role of the gut microbiome in the human immune response and could lead to better disease treatments down the line.
Imbalance of the gut microbiome has long been linked to disease. Ken Cadwell, an immunologist at the Perelman School of Medicine at the University of Pennsylvania, thinks of the microbiome as a metaphorical rainforest. “It’s a nice ecosystem—but if you cut down too many trees or bring in invasive species, you could make things go out of whack,” he says.
To see how the body’s internal fungi were affected during Covid and how this triggered the immune system, Iliev and his team started by looking at patients’ blood. After collecting samples from 91 people with Covid, they measured levels of antibodies against several fungi, to figure out if the body’s immune system was reacting against these. Significantly more anti-fungal antibodies, for instance, would indicate fungal overgrowth or invasion.
Takato Kusakabe, a postdoctoral fellow in Iliev’s lab and study author, ran plate after plate of experiments—a painstaking process—to quantify these antibody levels. The team found in patients with severe Covid, several fungi commonly found in the gut had increased antibodies against them (in comparison to uninfected people). Notably, these included Candida albicans, which is a common culprit of yeast infections. When the team then ran tests on fecal samples from 10 of the hospitalized Covid patients, these confirmed that the fungi being targeted by the antibodies were present in the patients’ guts—and at seemingly at higher levels than in uninfected controls, suggesting an imbalance in their microbiome.
The fungi in our guts can make cases of Covid worse Read More »